Research Description
The laboratory asks fundamental questions in bio-medical research. The focus of our research is morphogenesis, i.e. how cells are assembled into functional forms. We are concerned with the principles that determine the specific number, size and shape of different organs. This is an important process, as there is much to learn about the principles that can guide stem cells to form specific tissues and organs required for medical treatment. Our approach is to ask Nature how she does it – using the feather as a Rosetta stone to decipher these principles, because of the distinctive forms of feathers and interest in the evolution of flight. This unique model has allowed us to make several major impacts in the field of morphogenesis and develop interesting interfaces with scientists in different disciplines.
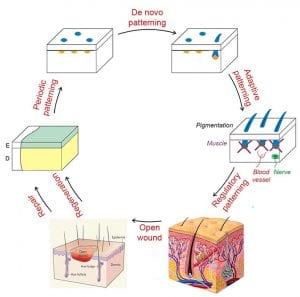
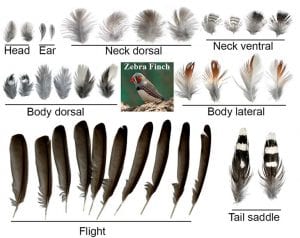
What’s new
“The making of a flight feather: Bio-architectural principles and adaptation” was published in the CELL Nov 27 issue and is also featured on the cover.
Cover caption: Birds have flight, contour, downy, and tail feather types, all of which serve different functions, with flight feathers conferring the ability to fly. A flight feather is made of two adaptable modules: the major shaft (rachis) and the vane. In this issue, Chang et al. study the molecular mechanisms of rachis and barb hooklet formation. Using quantitative biophysical approaches, they analyze feather rachis organization in birds with different flight characteristics and determine how multidimensional functionality can be achieved in the development and evolution of flight feathers for adaptation to different eco-spaces. The cover shows a photo of a Taiwan Blue Magpie with fully displayed feathers. Photo credit: Shao Hua Lang of Bird Photography Club in Taiwan.
Video abstract link (6 minutes) https://www.youtube.com/watch?v=YKuoVu4aOkg
Author list: Chang W-L, Wu H, Chiu Y-K, Wang S, Jiang T-X, Luo Z-L, Lin Y-C,Ang Li, Hsu J-T, Huang H-L, Gu H-J, Lin T-Y, Yang S-M, Lee T-T, Lai Y-C, Lei M, Shie M-Y, Yao C-T, Chen Y-W, Tsai JC, Shieh S-J, Hwu Y-K, Cheng H-C, Tang P-C, Hung S-C, Chen C-F, Habib M, Widelitz RB, Wu P, Juan W-T, Chuong C-M.
Abstract: The evolution of flight in feathered dinosaurs and early birds over millions of years required flight feathers whose architecture features hierarchical branches. While barb-based feather forms were investigated, feather shafts and vanes are understudied. Here, we take a multi-disciplinary approach to study their molecular control and bio-architectural organizations. In rachidial ridges, epidermal progenitors generate cortex and medullary keratinocytes, guided by Bmp and transforming growth factor β (TGF-β) signaling that convert rachides into adaptable bilayer composite beams. In barb ridges, epidermal progenitors generate cylindrical, plate-, or hooklet-shaped barbule cells that form fluffy branches or pennaceous vanes, mediated by asymmetric cell junction and keratin expression. Transcriptome analyses and functional studies show anterior-posterior Wnt2b signaling within the dermal papilla controls barbule cell fates with spatiotemporal collinearity. Quantitative bio-physical analyses of feathers from birds with different flight characteristics and feathers in Burmese amber reveal how multi-dimensional functionality can be achieved and may inspire future composite material designs.
Graphic Abstract:
- How does the avian intra-dermal muscle network form?
Networked structures integrate numerous elements into one functional unit, while providing a balance between efficiency, robustness, and flexibility. Understanding how biological networks self-assemble will provide insights into how these features arise. Here, we demonstrate how nature forms exquisite muscle networks that can repair, regenerate, and adapt to external perturbations using the feather muscle network in chicken embryos as a paradigm. The self-assembled muscle networks arise through the implementation of a few simple rules. Muscle fibers extend outward from feather buds in every direction, but only those muscle fibers able to connect to neighboring buds are eventually stabilized. After forming such a nearest-neighbor configuration, the network can be reconfigured, adapting to perturbed bud arrangement or mechanical cues. Our computational model provides a bioinspired algorithm for network self-assembly, with intrinsic or extrinsic cues necessary and sufficient to guide the formation of these regenerative networks. These robust principles may serve as a useful guide for assembling adaptive networks in other contexts.
Wu XS, Yeh CY, Harn HI, Jiang TX, Wu P, Widelitz RB, Baker RE, Chuong CM. Self-assembly of biological networks via adaptive patterning revealed by avian intradermal muscle network formation. Proc Natl Acad Sci U S A. 2019.
Intradermal muscle network is present in the skin of birds. (A) Schematic diagram showing region-specific dorsal-pelvic, femoral, and apteric tracts of chicken skin. (B and B′) Ventral and dorsal views of the 2D feather muscles show the criss-cross depressor and erector muscles (m.) attached to the tenascin domains. (C–E) Feather muscle patterns in dorsal-pelvic (hexagonal pattern), femoral (tetragonal pattern), and apteric (long and thin patterns) tracts at E12, stained with α-SMA (green), tenascin C (red), and DAPI (blue). Width of B is 500 μm. The dotted yellow line represents the midline (A-P). Two rows of muscle bundles connect along the A-P axis (yellow and red arrowheads). There are two kinds of lateral connections (anterolateral, white arrowhead; posterolateral, purple arrowhead) (n = 20). (D) Tetragonal configuration in the femoral tract; single muscle fibers connect with tenascin C domains on feather buds before the muscle bundle develops (green arrowheads) (n = 20). (E) Long and thin muscle fibers connect two distant feather buds in the apteric tract (golden arrowhead) (n = 20).
- How does the longitudinal pigment stripes on the quail skin form?
Animal skin pigment patterns are excellent models to study the mechanism of biological self-organization. Theoretical approaches developed mathematical models of pigment patterning and molecular genetics have brought progress; however, the responsible cellular mechanism is not fully understood. One long unsolved controversy is whether the patterning information is autonomously determined by melanocytes or nonautonomously determined from the environment. Here, we transplanted purified melanocytes and demonstrated that melanocytes could form periodic pigment patterns cell autonomously. Results of heterospecific transplantation among quail strains are consistent with this finding. Further, we observe that developing melanocytes directly connect with each other via filopodia to form a network in culture and in vivo. This melanocyte network is reminiscent of zebrafish pigment cell networks, where connexin is implicated in stripe formation via genetic studies. Indeed, we found connexin40 (cx40) present on developing melanocytes in birds. Stripe patterns can form in quail skin explant cultures. Several calcium channel modulators can enhance or suppress pigmentation globally, but a gap junction inhibitor can change stripe patterning. Most interestingly, in ovo, misexpression of dominant negative cx40 expands the black region, while overexpression of cx40 expands the yellow region. Subsequently, melanocytes instruct adjacent dermal cells to express agouti signaling protein (ASIP), the regulatory factor for pigment switching, which promotes pheomelanin production. Thus, we demonstrate Japanese quail melanocytes have an autonomous periodic patterning role during body pigment stripe formation. We also show dermal agouti stripes and how the coupling of melanocytes with dermal cells may confer stable and distinct pigment stripe patterns.
Inaba M, Jiang TX, Liang YC, Tsai S, Lai YC, Widelitz RB, Chuong CM. Instructive role of melanocytes during pigment pattern formation of the avian skin.
Proc Natl Acad Sci U S A. 116:6884-6890, 2019.
Quail feather pigment stripes
- Calcium oscillations coordinate feather mesenchymal cell movement by SHH dependent modulation of gap junction networks.
Synergistic actions of SHH-WNT gradients establish dermal gap-junction network, coupling dermal cells electrically to enable synchronized Ca2+ oscillations, which serve as a long-range signal relay mechanism to coordinate collective cell migration.
Li A, Cho JH, Reid B, Tseng CC, He L, Tan P, Yeh CY, Wu P, Li Y, Widelitz RB, Zhou Y, Zhao M, Chow RH, Chuong CM. Calcium oscillations coordinate feather mesenchymal cell movement by SHH dependent modulation of gap junction networks. Nat Commun. 9:5377, 2018.
- The birth of birds was selected as one of the top 10 scientific achievements of 2014 Science
We published a multi-disciplinary study integrating stem cell biology, tissue engineering, systems biology analyses, small molecule screening, and eventually a model on guiding multi-cellular configurations in a four-dimensional morphospace. Toward the application side, normally, many aging people become alopecic because adult cells gradually lose their regenerative ability. Here, with our new findings, we are able to make adult mouse cells produce hairs again! The work is also unique in that it applies time lapse movies to record cellular behaviors and analyzes the principles of how stem cells are organized into skin organoids.
Lei M, Schumacher LJ, Lai YC, Juan WT, Yeh CY, Wu P, Jiang TX, Baker RE, Widelitz RB, Yang L, Chuong CM. Self-organization process in newborn skin organoid formation inspires strategy to restore hair regeneration of adult cells. Proc Natl Acad Sci U S A. 2017 Aug 22;114(34):E7101-E7110.
- Multiple Regulatory Modules Are Required for Scale-to-Feather Conversion
We explored the evolution of scales to feathers by identifying a number of molecules using genomic analyses. Candidate genes were tested by expressing them in chicken and alligator scale forming regions. Ectopic expression of these genes induced intermediate morphotypes between feathers and scales which revealed several morphogenetic events along this path: localized growth zone formation, follicle invagination, epithelial branching, feather keratin differentiation and dermal papilla formation. We identified new molecules that act as scale to feather converters which induce one or more regulatory modules guiding these morphogenetic events. We propose that these morpho-regulatory modules were used to diversify archosaur scales and to initiate feather evolution.
Wu P, Yan J, Lai YC, Ng CS, Li A, Jiang X, Elsey R, Widelitz R, Bajpai R, Li WH, Chuong CM. Multiple regulatory modules are required for scale-to-feather conversion. Mol Biol Evol. 2017.
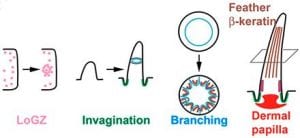
This paper has some news coverage. Here are links to the first two reports of this work. BBC news Eureka Alert
- Heterochronic truncation of odontogenesis in theropod dinosaurs provides insight into the macroevolution of avian beaks
We worked with Shuo Wang / Xing Xu, paleontologists in China on the evolution of beaks from dinosaurs. The work induced a lot of interest and is covered in the New York Times, Discover, etc.
Wang S, Stiegler J, Wu P, Chuong CM, Hu D, Balanoff A, Zhou Y, Xu X. Heterochronic truncation of odontogenesis in theropod dinosaurs provides insight into the macroevolution of avian beaks. Proc Natl Acad Sci U S A. 171: 427-439, 2017.
Macro-evolution of avian beaks
-
Genetic Mapping and Biochemical Basis of Yellow Feather Pigmentation in Budgerigars
We collaborated with Cooke / Bustamante in Stanford on a study of budgerigar colors . The paper is also covered by Nature and other news media. Budgerigars can have different coloring. Blue is based on the structural color and under different control. In this paper, we worked with the Stanford group to identify and characterize this chemical enzyme (polyketide synthase) which normally produces yellow pigment, but does not in the mutant enzyme form. When both are present, blue + yellow = green. The enzyme is already expressed in other tissues of the bird, but it is the co-optive expression in the axial plate that gives color to feathers. The axial plate is a special kind of feather epithelia that disappears to become space between feather branches. It is interesting that the enzyme is expressed in a special kind of keratinocyte instead of in melanocytes. This work is a multi-disciplinary effort.
Cooke TF, Fischer CR, Wu P, Jiang TX, Xie KT, Kuo J, Doctorov E, Zehnder A, Khosla C, Chuong CM, Bustamante CD. Genetic Mapping and Biochemical Basis of Yellow Feather Pigmentation in Budgerigars. Cell. 171:427-439, 2017.
Parrot pigment determination
The integument forms the interface between an organism and its environment. It serves diverse functions such as communication, endothermy, defense, and flight. During vertebrate evolution, various integumentary organs, including hairs, feathers, glands, and teeth, have evolved to help animals adapt to evolving environmental changes (1). These ectodermal organs form through epithelial-dermal interactions. Classic tissue recombination experiments have demonstrated that the dermis specifies the organ phenotypes within a developmental time window (2). Yet the underlying mechanisms remain unclear. (3) Here we reveal the specific molecular mechanism whereby an epithelial placode can be guided to form either a hair follicle for its architecture or a sweat gland for its secretory function.
Lai YC, Chuong CM. The “tao” of integuments. Science 354: 1533-1544, 2016
Organizing to adapt

- Self-organization process in newborn skin organic formation inspires strategy to restore hair regeneration of adult cells
Hairs grown from organic cultures
We used a multi-disciplinary approach integrating stem cell biology, self-organization cell behavior, tissue engineering, omics analyses, small molecule screening, and eventually a model on guiding multi-cellular configurations in a four-dimensional morphospace. Normally, many aging people become alopecic because adult cells gradually lose their regenerative ability. Here, we are able to make adult mouse cells produce hairs again! The work applies time lapse movies to record cell behaviors and analyze the principles of how stem cells are organized into skin organoids.
Lei M, Schumacher LJ, Lai Y-C., Juan W-T, Yeh C-Y, Wu P, Jiang T-X, Baker RE, Widelitz RB, Yang L, Chuong CM. Self-organization process in newborn skin organoid formation inspires strategy to restore hair regeneration of adult cells. Proc. Natl. Acad. Sci., USA. 114:E7101-E7110, 2017.
The movies shown in the paper were integrated from 500 movie recordings. The movies were taken using The USC BCC imaging core facility
USC news report on this paper
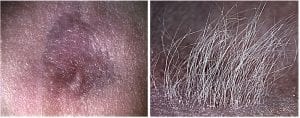
Skin cells from adult mice do not form hairs. With cues learned from the current study, the authors can environmentally reprogram dissociated skin epidermal (green) and dermal progenitor cells from adult mice to produce hairs through a series of morphological transitions to form a reconstituted skin after the skin organoids are transplanted onto nude mice.
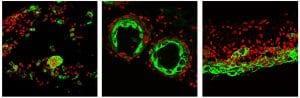